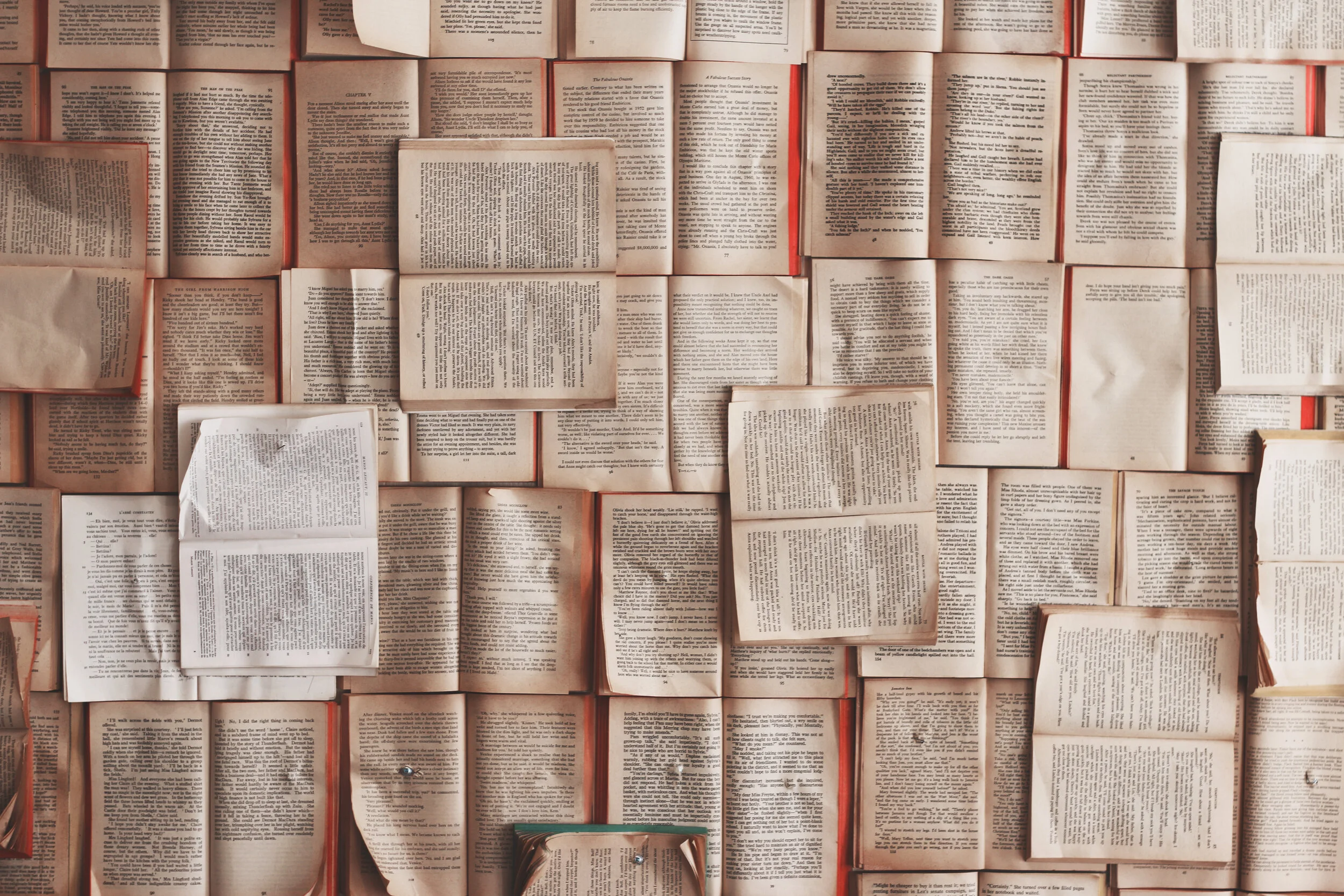
Articles
PART 6: Searching for Life Where the Sun Don't Shine: Explorations to the Seafloors of Earth and Europa
Part 6 of a 6-part series, originally produced as the thesis for my Master's from MIT's Graduate Program in Science Writing. Recently published in Astrobiology Magazine.
Steve Vance is still in the middle of planning discussions at JPL for a potential Europa mission that could launch as early as 2020. In early 2012, the team he’s on finished developing three different mission designs with three different vehicles—an orbiter, a flyby (like Voyager and Galileo) or a lander mission—that they’d pitched in a report to NASA agency brass the previous May.
Originally produced as the thesis for my Master's from MIT's Graduate Program in Science Writing. Recently published in Astrobiology Magazine.
This is the final part of a series that tells the story of humankind’s efforts to understand the origins of life by looking for it in extreme environments where life thrives, without relying on the Sun as an energy source. This series follows an oceanographic expedition to the Mid-Cayman Rise led by Chris German of the Woods Hole Oceanographic Institution, and NASA’s efforts to plan a future mission to Jupiter’s moon, Europa. By understanding how life can live without the Sun we may discover how life began on our planet, and whether or not Earth is the only place in the universe capable of supporting a biosphere.
Steve Vance is still in the middle of planning discussions at JPL for a potential Europa mission that could launch as early as 2020. In early 2012, the team he’s on finished developing three different mission designs with three different vehicles—an orbiter, a flyby (like Voyager and Galileo) or a lander mission—that they’d pitched in a report to NASA agency brass the previous May. At the time, the lander mission was determined to be too expensive and risky. Today, the agency is currently considering another flyby mission as the leading candidate to be Earth’s next ambassador to Europa.
Collecting samples on Europa and sending them back to Earth for laboratory analysis -- like German’s team does on Atlantis -- is probably many decades away from becoming a reality. Vance says a lander could collect samples, but it would be confined to collecting and analyzing only what it could reach at its landing spot. An orbiter or flyby mission, on the other hand, might be able to bounce radar waves off the icy surface to send back data about the moon’s mysterious ocean.
“If we had a mission with radar on it, you can construct a radar observation that could possibly see through more than ten kilometers of ice. Depending on how pure the ice is, radar propagates pretty well through ice,” says Vance. “There’s a possibility if (Europa has) a thin ice shell, you could see all the way to the ocean. At least to the extent that you could say, ‘here’s an ocean layer.’ You couldn’t see a whale…” Vance paused for a second, then clarified. “Not that we’re expecting to see a whale, per se.”

Image: One model of Europa’s ice. Some scientists think fresh seawater regularly cycles up to the surface of the moon through cracks, making exploration of the ocean more accessible to future landers. Credit: K. Kalousová
The mere thought that alien fishes, sharks and squids could be chasing each other around in the pitch black waters of Jupiter’s moon right now has astrobiologists like Vance itching to get a look below the ice. If there’s life on Europa, it’s much more likely to be microscopic bacteria — somewhat akin to what early Earth life was like for the vast majority of our planet’s biological history, some four billion years — but that doesn’t mean we can’t speculate.
Rock star communicator of astrophysics and space exploration, Neil deGrasse Tyson, agrees. “I want to go ice-fishing on Europa, cut a hole, put a submersible, look around, see if anything swims up to the camera lens and looks at the camera,” says Tyson.
One future mission to the Galilean moon that isn’t a part of NASA’s current plans yet could do just that. This concept is the cryobot: a robotic probe that could land on the surface of the ice, drill down through to the ocean by melting the ice using heat from its nuclear power source and then release a hydrobot (fancy term for robotic submarine) into the potential ocean below. The hydrobot would then begin collecting chemical measurements and start hunting for sea creatures.
Problem is, without detailed radar data of its surface, no one knows exactly how thick the ice layer is. Just as pizza aficionados debate the merits of New York thin versus Chicago-style deep-dish crust, so do planetary geologists argue over the thickness of Europa’s ice. According to Vance, there’s at least a mile and a half of ice at some points in Europa’s shell.
“Some of the craters have raised central peaks,” says Vance, a bit like if you imagine watching a commercial where a drop of milk falls in slow motion into a bowl, making a momentous splash, and you pause it right at the height of the upward splash. “In the case of these craters, the mechanics lead to the central peak freezing in place. And the central peak needs a certain amount of material underneath to support it.”
But that doesn’t necessarily mean you’d have to drill through all that ice to reach the ocean. Richard Greenberg, professor of planetary sciences at the University of Arizona, is an outspoken advocate for the New York-style thin crust ice layer. He thinks evidence from Voyagerand Galileo show that Europa’s geology cycles water up through the ice repeatedly, potentially bringing fresh seawater up to the surface and eliminating the need to drill through miles of ice to figure out if there’s life in Europa’s oceans.

Image: Artist's depiction of the recently shelved Jupiter Europa Orbiter (JEO) concept. Credit: NASA/ESA
“Everything on Europa’s surface came up from the ocean not long ago. If we were to land at a random place, we could hardly go wrong,” writes Greenberg in his book, Unmasking Europa. “If there is life on Europa, it may be hard to miss it.”
Ultimately, whether life can be found at the surface, below the surface, or not at all could depend on whether Europa’s geology supports similar types of volcanic gases mixing with transition metals and cold salt water, like the ROV Jason witnessed up close at the MCR hydrothermal vents. And even though we can get real-time photos and samples of vents on Earth, we still can’t observe anything about the mantle below the seafloor crust on our own planet, let alone a moon 500 million miles away.
“It’s an interesting parallel with the things that we don’t know very well on Earth,” says Vance. “Mantle convection on Earth is a big, difficult topic because we have to infer things indirectly from seismic data. We have to infer the structure and composition of the mantle from seismic observations.”
Planetary scientists apply similar inference techniques in determining the structure and composition of other planets and moons. To reconstruct the composition of Europa, Vance says “you apply the same physics that you’d apply to mantle geology to ice geology.” Observations to date have been able to give scientists a good idea of the rough magnitude of tidal energy that should be on Europa. But no one knows whether that energy gets dissipated in the ice shelf, the ocean or the rocky interior of the moon. “So there’s a partitioning problem which then relates to whether you can have underwater volcanoes,” says Vance. “It’s not as obvious.”
Tyson may have to wait awhile before packing up his ice fishing pole. Unfortunately for Vance and his colleagues at JPL, the agency’s planetary science budget just got hosed, to put it lightly, to the tune of a cool $300 million. These cuts to NASA’s 2013 budget (which has still not been approved at the time of this writing in January 2013) have forced the agency to renege on an agreement for joint U.S.-European robotic missions to Mars in 2016 and 2018, forcing the European Space Agency to scramble to find another partner—Russia. It’s also forced NASA to scale back ambitious missions to other planets and moons.

Image: Jupiter’s icy moon Europa. Credit: NASA
Such is the stark reality of planetary science in the 21stcentury in a country still recovering from a serious economic recession.
A shrinking budget is one reason Vance is part of the NASA team tasked with slimming down a previous proposed mission to Europa that would have placed an orbiter around the moon in 2026. With a planned launch in 2020, the very originally named Jupiter Europa Orbiter (JEO) would have spent 30 months touring the Jupiter system. The mission would have included four flybys of Io, nine of Callisto, six of Ganymede, and another six of Europa before entering a circular orbit around Europa for nine months, then ultimately meeting a fiery death in a controlled crash landing into the icy moon. The team had just finished its proposal when the planetary science Decadal Survey—a roadmap for figuring out priorities among thousands of planetary science mission proposals—came out. The survey brought mixed news. The good: Europa was deemed the second highest priority (behind Mars) for finding life in the solar system. The bad: the JEO mission was far too expensive at $4.7 billion to fund as it was originally designed.
Today, it seems that NASA’s next mission will be another flyby, but no one knows when or if Congress will approve the budget for such a mission. Meanwhile, other space agencies are already moving forward on their plans—the European Space Agency recently announced its next large space science mission will be the Jupiter Icy Moons Explorer — JUICE — to be launched in 2022. JUICE will arrive at the Jupiter system in 2030 and will focus on Europa’s neighbor Ganymede but will also include two flybys of Europa.
As for future missions, Vance is torn between the flyby and the lander concepts. Both have tradeoffs. He says Europa’s extremely high radiation levels are actually an advantage in the lander case. “It turns out you’d save a lot of money on operations for a lander mission,” said Vance. “The lander doesn’t live that long on the surface, because of the radiation environment. So it’s short and sweet. A lot of money that you spend on a mission is to keep a standing team of 100 people working on it for a long period of time. So a lander has [brevity] going for it.” That saves money.

Image: A possible scenario for life on Europa. Credit: Richard Greenberg
Despite savings in operational cost, higher initial up-front costs mean the lander mission will most likely have to wait for future funding opportunities. According to a May 2012 report from the Outer Planets Assessment Group (a NASA-sponsored forum for scientists and engineers to discuss plans for exploration of the outer solar system), the planetary science community favors either the flyby or orbiter mission options, given NASA’s current budget outlook. In particular, the group says that the flyby concept, with more than 30 close-range flybys of the moon, “offers the greatest science return per dollar, greatest public engagement, and greatest flow through to future Europa exploration.”
In addition, a flyby mission could yield a priceless result that could serve the whole scientific community for a long time: data.
“If you’re going to do this thing once every fifteen years, you want to get a whole, huge chunk of data that will keep you busy after your mission is over. And I can picture having detailed global mapping for geological and compositional interpretations as being that big chunk of data that you would want,” says Vance. These data could then be used to figure out an ideal landing spot for a future lander or cryobot mission.
Only getting one shot every fifteen years means the pressure’s on for Steve and his colleagues at JPL. He’s been studying Europa for about seven years. He’s 34 now. If a Europa mission — whatever it is — is launched during the proposed window for JEO, he’ll be 41. By the time that spacecraft makes it to the moon, he’ll be 47.
Last year before the Decadal Survey came out, he used to joke to friends and colleagues that if he was to father a child now, that child would have been born, taken a first step, learned to read, passed through adolescence, scared Steve to death at being behind the wheel for the first time, and well on his or her way to preparing for college all before the potential spacecraft made it to the Galilean moons.

Image: An artist impression of the The Jupiter Icy Moons Explorer. Credits: ESA/AOES
This is on the chance such a mission gets funded, launches successfully (on-time and on-budget), travels several billion miles through space using the gravity well of Venus as a slingshot, then lands (if they pick the lander) on the icy terrain of a radiation-soaked world that flexes by a hundred feet every 85 hours.
No wonder planetary scientists often think of their spacecraft as children: alive, young, naïve, full of potential, in need of protection, nourishment, guidance, and ultimately, deliverance.
******
Eight days after German and his crew reached the sunny Florida Keys, a team of Russian scientists finally reached the surface of Lake Vostok — a sub-glacial lake in Antarctica buried under 12,362 feet of ice. It took the team more than 15 years of stop and go drilling to reach the surface of the lake in the same spot that happens to hold the record for coldest temperature ever recorded on Earth: minus 89 degrees Celsius (minus 128 F).
The lake is roughly 14 million years old and has been completely untouched by humans for all of our history. It’s average temperature is calculated to be about minus 3 degrees Celsius and the only reason it remains in liquid form is because of the extremely high pressure caused by the weight of the ice above it. It has oxygen levels fifty times higher than most other freshwater lakes on Earth. It’s one of the largest lakes on the planet. It’s also completely cut off from the Sun.
But what could be most intriguing about this isolated lake is that it represents a bridge: a terrestrial environment that’s perhaps as close as we can get to studying an extraterrestrial one without leaving Earth. In other words, Lake Vostok is a bit like a mini-Europa ocean right here in our own backyard.
That the lake has been isolated for millions of years draws interesting questions about life, its origins and evolution. Is there life in the lake? If so, did it venture off on a completely different evolutionary branch than every other place on Earth? Could there be a heat source sustaining a chemosynthetic ecosystem like we find near hydrothermal vents in the oceans?
Just what’s down there?
By studying extraordinary environments like Lake Vostok, we’re building answers to these and other questions that help define life’s place in the universe. And that place is hardly defined yet. When confronted with the intricacies of life’s processes and the string of events that were required to create it, some speculate the origin of life is so improbable even on the scale of the entire universe that the only way it could have possibly arisen is if there exists an infinite number of universes, or a multiverse — ensuring the one we’re in had to have developed, eventually.

Image: Vostok Station. Credit: NOAA
Others, like Günter Wächtershäuser, argue that life’s not only likely to arise given the right starting conditions, but probably inevitable to emerge throughout the universe. “If this theory is in principle correct,” he says, “then life starts the same way everywhere—on Earth, or anywhere else in the universe. It starts with the same elements, with the same chemical properties of these elements and they will do the same reactions, with the same feedback and so forth.”
To Wächtershäuser, there is elegance in this predictability. “One day we will know how life starts and we will know that there is a chemical law for life’s beginning. It’s a chemical law. And we will know that as well as we know (that) the summation formula for water is H2O.”
As for Lake Vostok, the Russian team hasn’t been able to retrieve samples of the lake yet because their drill can only bring back ice samples, not liquid. They plan to return to the drill site early this year when the lake has frozen again over the Antarctic winter.
Meanwhile, Chris German is already busy planning his next two expeditions—he’s returning to the Mid-Cayman Rise this summer then taking what he’s learned there to the similarly ultra-slow-spreading, but also ice-covered, Gakkel Ridge in the Arctic Ocean (another great Earth-bound Europa analog) in the summer of 2014.
And Steve Vance is preparing the instruments that will be used, hopefully, to tell humanity whether or not we’re really alone in our own solar system.
And maybe, somewhere, a tubeworm will grow a little bit longer.
Waiting.
Part 5: Searching for Life Where the Sun Don't Shine: Explorations to the Seafloors of Earth and Europa
Part 5 of a 6-part series, originally produced as the thesis for my Master's from MIT's Graduate Program in Science Writing. Recently published in Astrobiology Magazine.
As he watched Florida recede in the distance, thoughts of the journey behind and the one lying before Chris German filled a hopeful mind. “Deep joy,” describes German, reflecting on his outlook at the start of the expedition.
Originally produced as the thesis for my Master's from MIT's Graduate Program in Science Writing. Recently published in Astrobiology Magazine.
This is Part 5 of a 6-part series telling the story of humankind’s efforts to understand the origins of life, by looking for it in extreme environments where life thrives without relying on the Sun as an energy source. It follows an oceanographic expedition to the Mid-Cayman Rise led by Chris German of the Woods Hole Oceanographic Institution, and NASA’s efforts to plan a future mission to Jupiter’s moon, Europa. By understanding how life can live without the Sun we may discover how life began on our planet, and whether or not Earth is the only place in the universe capable of supporting a biosphere.
As he watched Florida recede in the distance, thoughts of the journey behind and the one lying before Chris German filled a hopeful mind. “Deep joy,” describes German, reflecting on his outlook at the start of the expedition. “The mystery (of the existence of the vent sites) had already been removed by the 2009 & 2011 expeditions so we knew exactly where to go, I had the best kit on the planet to sample with in ROV Jasonand in my NASA/NSF team I had assembled some of the best experts in the world, who have also become my friends along the way, to take out and sample the site with,” says German. “It couldn't get much better.”
This voyage to the Mid-Cayman Rise (MCR) was planned to last 22 days. In 28 years of exploring since he began his PhD studies at the University of Cambridge, German has spent around a thousand days at sea, about on par for a modern day oceanographer. It’s a life of discovery and adventure but it also demands sacrifices—while at sea he’s missed his wife’s birthday (twice), his older son Martin’s 18th birthday, and his daughter Helen’s 16th birthday (while in the Antarctic).

Image: Jason returns from its last dive of the expedition. Credit: WHOI
Now on Atlantis, bearing down on potentially the most significant sampling of hydrothermal vent sites on the planet, German hoped real discoveries would be waiting for the team somewhere about 400 miles south of Havana.
In addition to grabbing temperature readings, biological samples and close-up shots of the vents, German and his colleagues wanted to study the MCR for what it can tell us about Earth’s history. Since the MCR is an ultraslow spreading ridge, it acts differently than its faster counterparts. Usually rocks get older the farther you go from the spreading center of a mid-ocean ridge. That’s because new seafloor is being created by magma erupting and then cooling and molding together with the older surrounding seafloor. But at ultraslow spreading ridges, the rate of magma generation doesn’t always keep pace with the rate at which tectonic plates are being pulled apart by subduction processes. These processes may be nearby or many thousands of miles distant at the far end of the plate, where older, colder, and more dense sections of ocean crust sink back into the underlying mantle.
Along key sections of the MCR, this movement of tectonic plates resembles a condition you may find on your bookshelf. Imagine removing a bookend to allow a row of books to tilt over on their sides. The upper face of a book cover that slides up and into view is like the rock that formed deep beneath the seafloor but has now slid up to be exposed at the seafloor where seawater-rock interactions can give rise to unusual hydrothermal systems. Importantly, even though these rocks were formed relatively recently in geologic time, they very closely resemble the compositions of the lavas that used to erupt at the seafloor back during the first 2-2.5 billion years of Earth history. Here we find a system in the modern world that may be very close to what hydrothermal systems were like during the brief but legendary lifetime of our good friend, Earth Life Form #1.

Image: Jason sampling a hydrothermal plume. Credit: WHOI
At 9:45 p.m. on January 8, Atlantis reached her destination above a hydrothermal vent site called Von Damm—named after the late geochemist Karen Von Damm. After two and a half days in transit, the ship rocked in the calm waves, keeping relatively stationary by use of one bow thruster and the stern propellers since her anchors couldn’t reach the seafloor, 7,500 feet below.
On the deck beneath two 42,000-pound capacity cranes sat the Remotely Operated Vehicle (ROV) Jason, named after an ancient Greek mythological hero famous for leading a group of adventurers called the Argonauts. Jason is the most capable U.S. academic research vessel roaming the seas today, able to reach 99% of the world’s seafloor at depths down to 21,000 feet.
The vehicle is also nearly indefatigable. It can work 24 hours a day with the help of a pilot, navigator, engineer, and three scientists. That means teams of six rotate through four-hour shifts, allowing Jason to remain submerged for many days at a time for continuous imaging, observation and sampling.
Tethered to Jason is it’s partner vehicle Medea, which serves as something of a shock absorber for the Greek hero. Any yanks on the line from Atlantis are transferred down to Medea, which maintains enough slack in the fiberoptic cable to allow Jason to roam around within 115 feet of Medea. (In Greek mythology, Medea was Jason’s wife.)

Image: Tubeworms. Credit: WHOI
Chris German is thankful for his robotic helpers and their human watchmen. He relies heavily on the engineering team for real-time adjustments to the dive plan and troubleshooting the Jason/Medea vehicles. Without the team’s constant attention to the robots, there are no photos of vents, samples of tubeworms, or up-close sonar mapping of the seafloor. He recalls a saying from Captain Dale of his 2009 expedition, who said, “Robots are like dogs: they’ll do their best, but neither one is any better than the person who trained them.”
The next day, the engineering team had Jason ready to go by noon, but a few technical difficulties pushed the inaugural dive back a few hours. By 4:30 p.m., Jason was on the ocean bottom with all systems running. Its first photos from the deep were of weathered rock outcrops, a few fish and shrimp, mussels and tubeworms. Data were displayed in an operations van—a mobile boxcar of screens and instrument displays for monitoring Jason’s progress that shared the deck of Atlantis with a host of equipment. Using its manipulator arms, Jason collected five tubeworms, a clam, and a sea cucumber.
The science teams started working their round-the-clock shifts. Twenty-three people from nine institutions in three countries made up seven science teams. There was the Carbon Team made of Max Coleman and Sarah Bennett from NASA’s JPL. They wanted to track the state of carbon as it exits from the vent sites and how it gets altered through the food chain. “We’re looking at the whole carbon cycle, from abiotic carbon production deep within the crust, to biotic carbon production through chemosynthesis,” says Bennett. “If hydrothermal systems were to exist on Europa, it’s studies like this that may help us to calculate the biomass that may exist.”
Just about everything seems to revolve around carbon. It’s an organic element, so the biologists study it because it tells you something about how life has evolved there. It’s preserved in rocks, so the geologists want to measure it to figure out the ages of their samples. It gets spouted from superheated vents, so the chemists want to know what it mixes with.

Image: View from the ROV operations van. Credit: WHOI
That’s why Coleman and Bennett are part of each of the other teams as well—the Plume, Biology, Geology, Fluids and Microbiology Teams—in order to get at the bigger picture of all things carbon in and around the vent systems.
After Jason’s first dive was declared a success, the crew ofAtlantis brought the ROV back up to the surface. SecuringJason and Medea to the deck and distributing samples among eager science teams, Atlantis made for the second target, named the Piccard vent site (named after late undersea explorer Jacques Piccard), merely two hours’ drive away.
******
By the time Jason submerged for its tenth and final dive of the expedition, Chris German and his team could already declare this mission a success. The team had returned to the Von Damm site for a final look at the rocks surrounding the hydrothermal system.
In the operations van aboard Atlantis, the Geology Team looked on—Guy Evans and Frieder Klein from Woods Hole and Matt Hodgkinson from the University of Southampton in the U.K. Unless you have the right rocks yielding the right chemistry, you won’t get the right conditions for life, regardless of what planet you’re on. At least as far as life as we know it goes. We’ve been wrong before.

Image: Geologist Frieder Klein from WHOI with a tough chunk of basalt. Credit: WHOI
“There are some conditions you need to understand when thinking about the origin of life," says Klein. "The key components are pH, hydrogen concentration, methane, CO2, and temperature, which we try to constrain using the rock record.”
A particularly interesting rocky outcrop north of the vent site appeared on the screens at around 9:00 p.m. The ROV pilot reached out with Jason’s robotic arms to grab hold of the rock and pull. The rock didn’t budge. He tried again, maneuveringJason’s arms to try a different angle. Still, nothing moved. After an hour-long struggle and with more biology and fluid samples to collect, Chris German called off the assault. The team dubbed the outcropping ‘kryptonite.’
By 4:00 a.m., Jason and Medea were still at work and Evans, Klein, and Hodgkinson came back to the operations van to resume the rock hunt after a brief rest. At 6:00 a.m. Jasonreturned to the area where it had wrestled with the “kryptonite”. A near-identical outcrop once again filled the screens and the team resumed the attack. Thirty minutes later, Jason’s strength was still no match for the rock. With another thirty minutes left of the team’s final dive, the Geology Team decided to move on to look for a more pliable sample.
Thus far, samples of mantle rocks—the ancient seafloor thought to be dragged up by the sliding-book-on-the-bookshelf tectonic plate at this ultraslow spreading ridge—had not been collected. If found, these rocks would help tell the story of the early ocean seafloor and, potentially, the environment surrounding the original genesis of life. The Geology Team wondered if Jason was wrestling with a primordial clue.

Image: Beebe vents 1 - 4. Credit: WHOI
Fifteen minutes before the end of the dive, the team found a third outcropping—similar in composition but slightly more weathered than the previous two. They decided to let their robotic friend engage in one final boulder brawl.
With three minutes left on the clock, Jason, leader of the Argonauts, broke free a chunk of the “kryptonite” the size of a Thanksgiving turkey-serving platter.
Back on deck, it didn’t turn out to be kryptonite at all! Only basalt. Tough, tough, layered basalt—a common form of volcanic deposit on the seafloor. Interesting, but not the clue they were looking for.
Maybe the sought-after mantle rocks were covered in sediment. Maybe they weren’t there after all. Or maybe they were still buried beneath the seafloor—shallow enough to interact with the circulating seawater but not exposed at the seabed. That, along with a continuous stream of unresolved questions reflecting the eternal curiosity and resiliency of the human spirit, will be up to future explorers to battle.
PART 4: Searching for Life Where the Sun Don't Shine: Explorations to the Seafloors of Earth and Europa
Part 4 of a 6-part series, originally produced as the thesis for my Master's from MIT's Graduate Program in Science Writing. Recently published in Astrobiology Magazine.
Nineteen seventy-seven was the kind of a year that only comes around once every 176 years. That’s how often the outer planets of our solar system (Jupiter, Saturn, Uranus, Neptune… sorry Pluto) line up just right in their orbits around the sun to allow for a spacecraft to slingshot past all four of them.
Originally produced as the thesis for my Master's from MIT's Graduate Program in Science Writing. Recently published in Astrobiology Magazine.
This is Part 4 of a 6-part series telling the story of humankind’s efforts to understand the origins of life by looking for it in extreme environments where life thrives without relying on the sun as an energy source. It follows an oceanographic expedition to the Mid-Cayman Rise, led by Chris German of the Woods Hole Oceanographic Institution and NASA’s efforts to plan a future mission to Jupiter’s moon, Europa. By understanding how life can live without the sun, we may discover how life began on our planet and whether or not Earth is the only place in the universe capable of supporting a biosphere.

Voyager spacecraft illustration. Credit: NASA.
Nineteen seventy-seven was the kind of a year that only comes around once every 176 years. That’s how often the outer planets of our solar system (Jupiter, Saturn, Uranus, Neptune… sorry Pluto) line up just right in their orbits around the sun to allow for a spacecraft to slingshot past all four of them. Rather than wait until 2153, NASA decided to take advantage of the opportunity of ‘77. The space agency launched the twin, 1-ton space probe emissaries, Voyager 1 and Voyager 2, from Cape Canaveral in September and August of that year, respectively.
Their mission was only supposed to last for four years, and technically, both spacecraft were only heading to Jupiter and Saturn. Mission designers had planned Voyager 2’s trajectory so it could continue on toward Uranus and Neptune only if Voyager 1 (which launched second but took a faster route) succeeded at Jupiter and Saturn. Fifty-two worlds and 12 years later, the Volkswagen Beetle-sized probes brought new meaning to success in planetary science.
Their journeys to the outer planets gave humanity a premier front row seat to see half the solar system up close in great detail for the first time (the Pioneer 10 and 11 spacecraft had zipped past Jupiter in 1973 and 1974, though they were far less capable and yielded significantly less data). The Voyager craft sent back stunning images of the Great Red Spot on Jupiter that revealed it to be a raging storm the size of two full earths. Saturn’s rings—thought to be smooth and ordered—were actually oddly intertwining and kinked. Uranus was orbiting sideways. Neptune owned the fastest winds in the solar system. And that was just the planets. The forty-eight moons captured by the Voyager craft were arguably even more striking than their parent planets.
The largest of Jupiter’s moons are the four Galilean moons—Io, Ganymede, Callisto, and Europa—so named for their discoverer, Italian astronomer Galileo Galilee. Galileo made the discovery in 1610 after tweaking his telescope to reach a magnifying capability of 20x (20 times better than the naked eye). These four worlds were the first moons discovered orbiting a planet other than Earth (since our own moon is awfully hard to miss, we’ll probably never know which of the first humans looked up and said, “what’s that?” Thus, it’s probably safe to credit Galileo with discovering moons).
The Galilean moons proved to be full of surprises at close range. An active volcano was spotted on the innermost of the Galilean moons, Io, spouting ash and gases a whopping 174 miles in height—the first evidence of active volcanism beyond Earth. The team would find half a dozen more erupting volcanoes on Io over the course of the mission. Team leader Brad Smith said this made Io look like a pizza. Later, astronomers would identify more than 400 active volcanoes there, making Io the most geologically active world in our solar system.
You’d think such a world might be a hot one, but you’d be wrong. Most of Io’s surface is -150 degrees Celsius. Colossal eruptions of gases condense and fall back to the surface as pastel yellow, orange and bluish white sulfur dioxide snow. This makes Io a surrealist’s dream: fiery hell plus winter wonderland with an everything-on-it sulfuric pizza (remember the egg smell?) surface and the churning clouds of Jupiter looming above hazy skies.
Finding Io’s volcanoes was like finding a smoking gun at a crime scene as far as astronomers were concerned—they disclosed a great deal of the moons’ mysteries right off the bat. The volcanoes determined Io’s swirling color palette—reds, yellows, browns, greens and gray-white sulfur dioxide frost. They also tipped scientists off to the phenomenon of tidal heating, which is the cause of Io’s agitation and the source of hope for testing Wächtershäuser’s hydrothermal vent theory beyond our home planet.
As previously mentioned, volcanic activity on Earth is produced by a heat source that comes from energy released from the decay of radioactive materials deep inside the planet and also leftover heat from its formation. But Io is too small for leftover heat and too active to explain its volcanoes by radioactive decay alone. Instead, the strong gravitational force from Jupiter pulls Io inward, while the weaker gravitational forces of its neighboring Galilean moons, Europa and Ganymede, pull Io outward. These three moons actually fall into an elegant, stabilizing dance coined orbital resonance, where Io travels around Jupiter exactly twice as fast as Europa, which travels twice as fast as Ganymede. This makes the distance between Io and Jupiter vary along its orbit as the moon fights with the competing forces (or tidal forces). These tremendous tidal forces alternate between squeezing and stretching its core, causing Io’s surface to rise and fall by the length of a football field every time it completes an orbit, which it does every 42.5 hours. This squeezing and stretching causes friction. Friction causes heat and pressure. Heat and pressure cause molten material and gases to explode from the surface. Hence, volcanoes.
Io’s smoking gun was pointing straight at its neighbor, the next closest Galilean moon to Jupiter: Europa. At the time of Voyager 1’s flyby of Io on March 5, 1979, scientists had just begun to re-think the internal makeup of the Galilean moons. If there was tidal heating on Io, partially caused by gravitational forces of Europa and Ganymede, wouldn’t there be similar forces acting on Europa and Ganymede, too?
A month after the Io flyby, Voyager 1 came within several hundred thousand miles of Europa, though its trajectory was planned to take it back around Jupiter and away from the moon. Scientists had to wait another four months for Voyager 2 to get up close to Europa.
By the time Voyager 2 reached Europa, the team’s hopes were soaring. They knew Europa was rich with water in its icy outer layer. If there was tidal heating on Europa, could it be enough to melt the bottom of the moon’s ice crust, perhaps enough—gasp—to sustain a liquid water ocean?
Some even imagined they’d see several miles-long geysers bursting from the icy shell. But when the first photos came in, the team found no geysers. Instead, they were confronted with a giant white eyeball staring back at the camera, striped with wide brown veins slicing across the entire surface.
If you were to walk across that surface, Europa’s stripes would appear almost painted on. There’s almost no elevation change across most of the moon. Some scientists think the stripes may be cracks in the ice that spread pieces of the crust apart—not unlike seafloor spreading on Earth—allowing for liquid water to well up from the ocean beneath and freeze again, forming a younger swath of icy surface.
Despite the absence of geysers or volcanoes on its surface, Europa does feel strong tidal forces. On Earth, the tidal force from the moon controls our ocean tides, which influence when ships leave for a day of fishing or whether a sand castle on the beach will meet its demise an hour after its construction. But on Europa, the effects of its host planet are a bit steeper. Jupiter is so much bigger than our moon—30,000 times more massive—and so close to Europa that its tide stretches the 2,000-mile wide moon by more than100 feet. This also makes the moon’s orbit ever-so-slightly non-circular, giving the orbit a tiny eccentricity, but one with enormous implications. Huge tidal forces are the crucial factors that suggest Europa’s ocean is able to remain in its liquid water phase instead of freezing completely into the layer of ice above it. According to planetary scientists, everything interesting about Europa follows from this subtle eccentricity.
Further evidence for liquid water on Europa came from the Galileo mission, launched in 1989, which brought far greater scientific firepower to bear on Jupiter and its moons. In particular, Galileo measured Europa’s magnetic field and found that it flips direction every five and a half hours—another byproduct of the domineering influence of its host planet.
If an electrical conductor is placed in a varying magnetic field, electrical currents will be induced in it. Those currents will then induce a magnetic field oriented in the opposite direction to the original magnetic field that generated the current, partly cancelling it out. Since Jupiter has a powerful magnetic field and its axis of rotation doesn’t exactly line up with its magnetic axis, Europa crosses from the north side to the south side of Jupiter’s magnetic field—or vice versa—every five and a half hours. Imagine you are Jupiter and Europa is orbiting around your belt. Now, imagine trying to learn to hoola hoop for the first time. The wobbly hoop is the orbit of Jupiter’s magnetic field—and every time it touches your belt, it’s wobbling in a different direction. Up, down, up, down, up, down. This causes the orientation of the magnetic field in Europa’s orbit to vary as Jupiter rotates.
In Europa’s case, a magnetic field oriented in the opposite direction to Jupiter’s would indicate the moon has a massive electrical conductor somewhere inside it. And it just so happens that a moon-swallowing subsurface saltwater ocean matches the prediction for this electrical conductor quite nicely.
Along with its two compatriots Io and Ganymede, Europa dances a violent, synchronized tango around its host, causing friction and inducing a magnetic field—enough, some scientists hope, to indicate the presence of a sloshing ocean somewhere beneath miles of occasionally shearing ice.
Below that potentially 100-mile deep ocean, there may be a seafloor that’s not so different from our own.
PART 3: Searching for Life Where the Sun Don't Shine: Explorations to the Seafloors of Earth and Europa
Part 3 of a 6-part series, originally produced as the thesis for my Master's from MIT's Graduate Program in Science Writing. Recently published in Astrobiology Magazine.
Hydrogen sulfide is a poison gas that’s lethal for humans even in very low concentrations. Yet, this compound—two parts hydrogen, one part sulfur—turned out to be the food source for bacteria that were driving an entirely new ecosystem.
Originally produced as the thesis for my Master's from MIT's Graduate Program in Science Writing. Recently published in Astrobiology Magazine.
This is Part 3 of a 6-part series telling the story of humankind’s efforts to understand the origins of life by looking for it in extreme environments where life thrives without relying on the sun as an energy source. It follows an oceanographic expedition to the Mid-Cayman Rise, led by Chris German of the Woods Hole Oceanographic Institution and NASA’s efforts to plan a future mission to Jupiter’s moon, Europa. By understanding how life can live without the sun, we may discover how life began on our planet and whether or not Earth is the only place in the universe capable of supporting a biosphere.

Image: Origin of Life on Earth. From left to right, starting from a volcanic primitive Earth and simple molecules in the ocean to complex life forms on land. Credit: NASA
Hydrogen sulfide is a poison gas that’s lethal for humans even in very low concentrations. Yet, this compound—two parts hydrogen, one part sulfur—turned out to be the food source for bacteria that were driving an entirely new ecosystem. (New to us at least. Some scientists suspect this type of ecosystem might, in fact, be the oldest type of ecosystem on our planet.)
For more than a century, biologists have known that bacterial life can exist based on chemosynthesis, but before the 1977 Galapagos Hydrothermal Expedition, no one had imagined an entire ecosystem could be generated from chemosynthetic processes alone.
Chemosynthesis is the biological conversion of carbon molecules and nutrients into organic matter—the stuff of life. Whereas photosynthesis uses energy from sunlight to convert carbon dioxide into that organic matter, giving off oxygen as a byproduct, chemosynthesis uses inorganic molecules (such as hydrogen sulfide) or methane and combines them with an oxygen source (in this case seawater) to create simple sugars. Those sugars are the fuel that bacteria live off and the bacteria are the fuel that larger organisms need to survive. Chemosynthesis that uses hydrogen sulfide produces sulfur as a byproduct—and it’s the sulfur that smells like rotten eggs.
Hydrogen sulfide is one of many compounds that hitch a ride in the superheated water as they rocket back up to the surface of the seafloor after sneaking down through fissures to meet pockets of magma from the Earth’s mantle. These substances get dissolved by the hot water from the rocks lining the fissure, creating a chemically-enriched water mixture. This mixture then gets buoyant as it’s heated, eventually escaping back into the frigid ocean water.
But how could such an alien process—fundamentally different from the basis for the vast majority of all life on Earth—exist here?
Enter Günter Wächtershäuser. A German chemist turned patent lawyer who specializes in chemical and biochemical inventions, Wächtershäuser (pronounced VEK-terz-hoi-zer) first proposed in 1988 that a similar chemosynthetic process to the one observed on the Galapagos Hydrothermal Vent Expedition had been around on Earth for a very long time. Not only was it common to the planet Earth throughout its history, he argues, this process was fundamental to all living ecosystems going back to the very first genesis of life around four billion years ago.
If we could travel back to that time, we’d see a wholly different Earth than we see today. We’d see a planet emerging from the cataclysm of an impact with a rogue planet that created the Moon and is thought to have tilted the Earth’s axis to about 23.5 degrees. The planet would have only recently cooled down enough to bear a solid crust, though intense volcanism and geological upheaval would have created a toxic atmosphere almost completely devoid of oxygen. With no ozone layer, the planet would be drenched with massive doses of ultraviolet light and radiation from the sun. We’d see comets, meteorites and asteroids raining down across the landscape in a period of heavy bombardment, leaving behind condensed water that would form clouds and eventually, combined with volcanic outgassing, oceans. Photosynthesis wouldn’t take place on Earth for perhaps another two billion years. Yet, it would have been in this literal hell on Earth where we would have seen (if we’d remembered to take a microscope with us) the first life forms start to appear.
Wächtershäuser, who’s also an honorary professor for evolutionary biochemistry at the University of Regensburg in Germany, points to Earth’s early oceans and period of high volcanic activity as the perfect environment for the birth of Earth Life Form #1, or the “pioneer organism”, an acellular (containing no cells) entity that likely passed through the loneliest life in the history of the planet in a manner of minutes. His origin of life premise is called the Iron-Sulfur World Theory. It says that hot, pressurized water mixed with dissolved gases (including hydrogen sulfide, carbon monoxide, carbon dioxide, hydrogen cyanide and ammonia) passed out of prehistoric vents and over various minerals containing iron, nickel and other metals within the rocks around the vents. These metals served as catalysts for a chain reaction that synthesized organic compounds and coupled some of them with other metals to form new compounds with greater ability to yield new chemical reactions.
This coupling between the catalyst and the product of an organic reaction is the key first step of Wächtershäuser’s theory.
What comes next is the miracle of evolution. Starting with these metals and gases reacting together as life emerged, Wächtershäuser says evolution starts with the beginning of a primitive metabolism that created increasingly complex chemical reactions, eventually leading over time to the formation of DNA—life’s blueprints for making more living cells today. Before living cells were around, these metals and gases reacted together in a purely chemical sense, according to predetermined “pathways.” There were only so many compounds around back then and only so many ways these could react together. “Hydrogen sulfide, sulfur dioxide. Hydrogen cyanide, carbon monoxide, carbon dioxide, hydrogen, nitrogen, ammonia,” says Wächtershäuser, pausing before adding “Oh, phosphor oxide, P4O10. That’s it.” Aside from a few other trace molecules, these were the gases belched from the belly of the planet that formed the first chemical reactions that served as the precursor to life.
When these gases come up from deep within the Earth, they move from a state of high pressure, high temperature to one of low pressure, low temperature. This happens rapidly, causing a non-equilibrium condition called quenching—where the ratio of gases at a high temperature gets frozen in the same concentration upon transferring into a low temperature environment. “Let’s say at high temperatures you have an equilibrium ratio of carbon dioxide to carbon monoxide, 1:1,” says Wächtershäuser. “And at low temperatures, you may have an equilibrium ratio of 10,000:1. So if you quench, then you may have a low temperature mixture still having a high concentration of carbon monoxide.”
The quenching is important because it creates a chemical potential. The chemical potential drives the reactions between gases and metals at hydrothermal vents. Here, because of the unique combination of the right gases and the right metals at the right temperatures, reactions start to build off each other, creating “a synthetic reaction whereby the organic products that are synthesized promote in turn the rate of another reaction,” says Wächtershäuser.
In other words, certain reactions create byproducts that then are used to speed up other reactions, leading to longer, more complex strings of organic molecules.
According to his theory, all evolution happens using this method of “feed forward” reactions, where organic products promote other reactions yielding new organic products of increasing complexity. This is what gave rise to amino acids, peptides, sugars, proteins, nucleotides, and nucleic acids, like DNA and RNA. After that, as the theory goes, life evolved higher levels of complexity and ventured beyond vent communities. It isn’t clear when photosynthesis first arose, but most estimates range from 2.5 to 3 billion years ago. The rest, as they say, is history.
Since we can’t go back in time, we’ll never know if Günter’s theory of life rising from the depths of the Earth’s molten innards is true or not. And his is only one of several competing origin of life theories, which generally fall into two camps. Metabolism-first theories (like the Iron-Sulfur World Theory) start with simple molecules that build increasing levels of complexity. Replicator-first theories, on the other hand, suggest that simple organic molecules occur naturally and are able to self-replicate right from the start.
Chemist Stanley Miller is in the replicator-first camp. Miller, who is sometimes referred to as the “Father of origin of life chemistry,” earned early fame for a classic experiment he conducted in 1952 as a graduate student with Harold Urey demonstrating how amino acids could be generated in a lab environment from simple compounds subjected to electrical discharges in the early Earth atmosphere. The spontaneous creation of amino acids doesn’t by itself explain life’s origins, since it’s still a huge jump to go from simple amino acids to complex, self-replicating chains of genetic instructions contained within DNA and RNA. But his findings did lay the cornerstone for origin of life research and sparked many curious minds to test all sorts of combinations of conditions that could have been around on the early Earth. Still, sixty years later, none of these efforts have been able to replicate life from scratch in a lab.
One of the leading variants of the replicator-first camp is the “RNA World Theory,” which suggests that RNA molecules arose before the first proteins since before them, there would have been no molecule of heredity, or “blueprint” molecule to manufacture other molecules consistently. Advocates of this theory say there was a time when RNA alone handled all maintenance activities of a cell, acting as both genetic material and a catalyst for metabolism reactions. The evolution of DNA then reallocated labor for replication and genetic storage to the more stable DNA molecule.
But Wächtershäuser thinks it’s too much of a jump to assume life began with the ability to self-replicate. “The beginning of the genetic machinery is not replication. Replication has no purpose in itself,” says Wächtershäuser. To him, the beginning of the genetic machinery of replication begins with something akin to the chemistry that goes on in the core of the ribosome, which is a bit like the manufacturing plant of all cells. That chemistry boils down to peptide formation—basically, simple amino acids linked together. It’s a crucial step and it’s something his experiments have been able to produce in the lab under high temperature conditions—the same conditions that are thought to have been around in the early Earth around hydrothermal vent systems. It isn’t conclusive proof his theory’s right, but he’s getting closer.
Unfortunately for Wachtershauser, maybe the best proof to his theory lies about 500 million miles away from his lab in Germany, beneath the icy shell of a moon discovered more than 400 years ago by a man named Galileo Galilei.
PART 2: Searching for Life Where the Sun Don't Shine: Explorations to the Seafloors of Earth and Europa
Part 2 of a 6-part series, originally produced as the thesis for my Master's from MIT's Graduate Program in Science Writing. Recently published in Astrobiology Magazine.
Just as it helps Chris German answer riddles about the origin of life on Earth, life’s surprising hardiness gives astrobiologists cause for hope in finding life on seemingly inhospitable hells off Earth, too. Among researchers on the search for those hells is Steve Vance, a member of the Science Definition Team for NASA’s Europa missions.
Originally produced as the thesis for my Master's from MIT's Graduate Program in Science Writing. Recently published in Astrobiology Magazine.
This is Part 2 of a 6-part series telling the story of humankind’s efforts to understand the origins of life by looking for it in extreme environments where life thrives without relying on the sun as an energy source. It follows an oceanographic expedition to the Mid-Cayman Rise, led by Chris German of the Woods Hole Oceanographic Institution and NASA’s efforts to plan a future mission to Jupiter’s moon, Europa. By understanding how life can live without the sun, we may discover how life began on our planet and whether or not Earth is the only place in the universe capable of supporting a biosphere.

Image: Artist’s representation of two scenarios of what may be happening under the ice on Jupiter’s moon Europa. Credit: JPL/NASA
Just as it helps Chris German answer riddles about the origin of life on Earth, life’s surprising hardiness gives astrobiologists cause for hope in finding life on seemingly inhospitable hells off Earth, too. Among researchers on the search for those hells is Steve Vance, a member of the Science Definition Team for NASA’s Europa missions. As Chris German and the crew of Atlantis set out to scoop up evidence for life on the bottom of Earth’s seafloor, Vance met with a team of engineers and planetary scientists at NASA’s Jet Propulsion Laboratory (JPL) to plan a mission with a similar objective yet radically different destination. Their goal: to find out if there’s hydrothermal vent-like life on Jupiter’s moon, Europa.
Vance and his colleagues are busy planning a mission to send a robotic spacecraft to gather more data on the icy moon to determine whether life once existed (or still exists today) in an ocean below the surface-covering layer of ice there. Based on data from Voyager and, later, the Galileo robotic flyby missions, scientists think there could be similar geologic processes on Europa’s seafloor as we find on our own seafloor. If so, there’s reason to believe life could be thriving today in the cold, dark depths there, huddled around oases of hydrothermal vents just like on Earth. That’s why, to people like Vance, it’s at the intersection of life where space and ocean exploration come together.

Traditionally, the search for life beyond Earth has been focused on a region surrounding the sun (or in the case of other solar systems, another star) where a planet or moon with sufficient atmospheric pressure can maintain liquid water on its surface. This is based on the assumption that life elsewhere beyond Earth would be a lot like life found on our planet—particularly, that liquid water would be essential to its survival. The search for the right conditions for life involves finding a planet or moon in a location that isn’t too close to its host star to boil off any liquid water or too far from the star to keep all water in its solid ice phase. This is what astrobiologists and astronomers refer to as a “Goldilocks planet”—one that’s not too big, not too small, not too hot, and not too cold—but just right.
Except, Europa isn’t a Goldilocks planet. It lies well outside the supposed habitable zone of our solar system (which conveniently only includes a region of space containing Earth and our moon). So how could life possibly survive on Europa?
The answer lies in how life gets its energy. More than 99% of life on Earth gets its energy from the sun. Plants get their energy from photosynthesis, which produces oxygen. Animals breathe this oxygen and eat the plants. Other animals eat those animals. Then animals die and become nutrients in the soil for plants, thus completing the extraordinary, miraculous circle of life as we know it.
But hydrothermal vents demonstrate that not all life needs the sun to serve as the stork. The remaining less than 1% of life just needs a little bit of superheated water cooked up by rocks deep within the earth to generate the right blend of rotten egg-smelling fluids that can then be combined with near freezing seawater at bone-crushing depths to allow them to survive.
Simple enough, right?
The process of obtaining energy from chemical reactions between water and the young underlying seafloor rather than sunlight is called chemosynthesis. In the case of hydrothermal vents, the primary compound is hydrogen sulfide. This chemical process is the reason why NASA’s interested in hydrothermal vents on Earth’s seafloor. And if chemosynthetic life exists anywhere outside of Earth, Europa would be a good place to start looking for it. The sixth closest of Jupiter’s moons, Europa may be one of the few places in our solar system where chemosynthetic life not only could have lived in the past, but might still be thriving today. If there’s water and the moon is as thermally active as scientists think, the conditions might be just right to sustain an alien ecosystem.
Vance and his colleagues at JPL are hoping to be the first to find that alien ecosystem. They’re currently working on a proposal to launch a mission to Europa in 2020, which could reach the icy moon by 2026. It’s a long time to wait, but such is the reality of conducting research on a subject 500 million miles away.
This is where the explorations of the very high meet those of the very low. And there may be no greater connection—or common purpose—between exploration of the heavens and the depths of our seas than in the search for life and its mysterious origins.
******
Before 1977, biology textbooks claimed that to sustain a living ecosystem, you needed energy from the sun. That was before Donnelly, van Andel, and Corliss dove to the bottom of the sea in Alvin as part of the Galapagos Hydrothermal Vent Expedition.
Their mission didn’t have anything to do with rewriting biology textbooks. Van Andel, a geo-archaeologist, and Corliss, a geologist, were more interested in the seafloor itself. Scientists had speculated the existence of hydrothermal vent systems, but no one had ever proven they were there and certainly no one had seen them in person. Their speculation stemmed from heat-flow calculations along the 42,000-mile global mid-ocean ridge system—a planet-wide mountain range that forms the largest geographic feature on Earth and snakes around the seafloor like the seams on a baseball. This underwater mountain range marks the line where Earth’s tectonic plates are spreading apart from each other—the same phenomenon behind the suspiciously similar coastlines of South America and Africa that make the jigsaw-like continents appear to have fit snugly together at one time. Well, they did. That is, before seafloor spreading pushed them apart over millions of years at a rate about as fast as your fingernails grow, creating gaps in the ocean’s bottom along the ridge where new crust from the planet’s mantle oozed upwards, cooled, and formed brand new seafloor.
Scientists made heat-flow calculations to characterize what this new crust should look like. Clive Lister, a geophysicist at the University of Washington, argued that since the young crust coming up from the mantle was being cooled by the near-freezing ocean water, it should cool and contract the farther it got from the ridge. That meant the hottest spots should be near the ridge. Yet, when actual heat-flow measurements were made at portions of the seafloor up to several hundred miles out from the ridge, everything looked right except for those spots nearest the ridge. They weren’t as hot as they should have been. Where was the missing heat?
Lister speculated that the ridge was essentially a giant heat blister. Molten rock chambers at temperatures of 1,200-1,400 degrees Celsius lay below the ridges and cracks in the seafloor allowed cold water to seep into the crust. Where cold water met hot magma, the water expanded, became superheated and shot back up to the surface in the form of underwater hot springs, or hydrothermal vents. This is where he thought the missing heat might be going.
At least that was the going theory as Donnelly steered Alvin down into the darkness. He, van Andel, and Corliss were on the search for missing heat.
Enclosed in an almost seven-foot diameter titanium pressure sphere, the three men huddled together behind walls less than two inches thick. At a depth of 9,000 feet, they had long since passed the point where the suns rays penetrate the sea (roughly 3,300 feet). The water pressure at this depth is nearly 300 times higher than at sea level, which meant that every square inch of the hull felt about the same as your big toe would feel with the weight of an entire Jeep Wrangler pressing down on it. “If seawater with that much pressure behind it ever finds a way to break inside, it explodes through the hole with laser-like intensity,” wrote legendary oceanographer Bob Ballard in The Eternal Darkness. Ballard was the co-chief scientist of the Galapagos Hydrothermal Expedition (along with Richard von Herzen) and was onboard the support ship, Knorr, while Donnelly patrolled the seafloor in Alvin. “A human body would be sliced in two by a sheet of invading water, or drilled clean through by a narrow (even a pinhole) stream, or crushed to a shapeless blob by a total implosion,” wrote Ballard.
The crew relied completely on Alvin’s quartz iodide and metal halide lights to illuminate their path through the darkness. To navigate, Donnelly used three transponders—which the team named Sleepy, Dopey, and Bashful—that had been dropped five days earlier in a triangular pattern at various points in the area. Unlike airplanes, which use light and radio waves to navigate, submersibles need to rely on sound waves (just like dolphins) to figure out where they are and where they’re headed. Light and radio waves can’t travel very far in water. Alvin’s navigation computer sent out sound waves toward the transponders, which in turn, sent back sound waves of their own. Based on the data from the transponders, Donnelly was able to steer Alvin toward the team’s target location on the seafloor.
Their intended target had been identified two days earlier, when the Knorr had located a spike in water temperature near the seafloor. The research vessel had been dragging a camera sled called ANGUS (Acoustic Navigated Geological Undersea Surveyor) outfitted with a sensitive thermistor capable of picking up tiny temperature changes in the water. Operators on Knorr needed to keep ANGUS 15 feet above the seafloor—a difficult task given the varied landscape of the rugged, volcanic terrain.
Good thing ANGUS’s toughness matched the motto on its side: “Takes a Lickin’ But Keeps on Clickin’.” Despite occasional collisions, operators were able to maneuver the 2-ton steel sled over seven miles of seafloor real estate in a 12-hour span. Only one three-minute period of trawling had yielded anything significant.
When the team reviewed the photos the next day, they were shocked at what they saw. Thirteen photos taken during that three-minute interval revealed an incredible accumulation of life—mostly in the form of white clams and brown mussel shells—that no one had expected to see. Living communities this deep had never been seen before. The deep ocean floor was supposed to be devoid of life.
Van Andel and Corliss sat with eyes peeled out the sub’s 4.5-inch circular viewports. Not only were they looking for heat in a cold, barren abyss; they were now on the search for life.
As Donnelly zeroed Alvin in on their target, formations of long-cooled, hardened lava “pillows” were all they could see. These had been formed as cracks emerged in the crust, caused by the seafloor spreading. The cracks allowed magma to spew from the Earth, cool, and form mounds as if the planet had squeezed hundreds of tubes of toothpaste from its belly and failed to utilize any of it.
Alvin inched closer and the team noticed the lava patterns began to change—appearing smoother and shinier as they approached the target. These lava flows were sinewy and suggestive of faster, fresher flows. They were getting closer.
When at last they reached the coordinates of the temperature spike, the crew entered an alien world. The dark water shimmered blue from manganese and other minerals carried up through the crust by superheated water. Clams measured a foot or more in length piled high surrounded by shrimp, crabs, fish, and small lobster-like creatures. Strange plant-like organisms grew from the rocks, appearing like dandelions, and bizarre, wormy tendrils reached out from clumpy harbors.
“Isn’t the deep ocean supposed to be like a desert?” Corliss asked a graduate student on the support ship Lulu by acoustic telephone.
It was. Until now.
Not only had the team found their missing heat by discovering the first hydrothermal vents, but they’d also stumbled upon something else, something potentially even more profound. “A suspicion dawned on us,” reported Ballard. “These unexpected life forms might actually be a bigger discovery than the expected warm water.”
When Donnelly brought Alvin back to the surface, the research team struggled to figure out what to do with their unexpected biological samples. They hadn’t prepared for living samples. They thought they’d be dealing with rocks. They didn’t even have a single biologist onboard (only geologists, geophysicists, chemists, geochemists, physicists, and one lucky science writer).
A small amount of formaldehyde and some Russian vodka the team had picked up in Panama were the only preserving liquids they could come up with. Over the next several days, the team found four more vent sites, each unique from the previous one. They came up with names for each site—Clambake I, Clambake II, Oyster Bed, Dandelion Patch, and, finally, the Garden of Eden.
The discovery of these sites was applauded throughout the scientific community. Biologists were eager to find out just what sort of life could live in darkness on the bottom of the ocean. Hydrothermal vents and the ecosystems they generated would come to redefine the making of the planet and our theories about how life began here. But first, there was the issue of the rotten eggs smell.
PART 1: Searching for Life Where the Sun Don't Shine: Explorations to the Seafloors of Earth and Europa
Part 1 of a 6-part series, originally produced as the thesis for my Master's from MIT's Graduate Program in Science Writing. Recently published in Astrobiology Magazine.
This series tells the story of humankind’s efforts to understand the origins of life by looking for it in extreme environments where life thrives without relying on the sun as an energy source. It follows an oceanographic expedition to the Mid-Cayman Rise, led by Chris German of the Woods Hole Oceanographic Institution and NASA’s efforts to plan a future mission to Jupiter’s moon, Europa. By understanding how life can live without the sun, we may discover how life began on our planet and whether or not Earth is the only place in the universe capable of supporting a biosphere.
R/V Atlantis, morning before departure to the Mid Cayman Rise. Image Credit: Woods Hole Oceanographic Institution (WHOI)
On February 17, 1977, Tjeerd van Andel of Stanford University and Jack Corliss of Oregon State took a few last breaths of the South Pacific air before closing the basketball hoop-sized hatch of the research submersible, Alvin.
Originally produced as the thesis for my Master's from MIT's Graduate Program in Science Writing. Recently published in Astrobiology Magazine.
This is Part 1 of a 6-part series telling the story of humankind’s efforts to understand the origins of life by looking for it in extreme environments where life thrives without relying on the sun as an energy source. It follows an oceanographic expedition to the Mid-Cayman Rise, led by Chris German of the Woods Hole Oceanographic Institution and NASA’s efforts to plan a future mission to Jupiter’s moon, Europa. By understanding how life can live without the sun, we may discover how life began on our planet and whether or not Earth is the only place in the universe capable of supporting a biosphere.

Image: R/V Atlantis, morning before departure to the Mid Cayman Rise. Image Credit: Woods Hole Oceanographic Institution (WHOI)
On February 17, 1977, Tjeerd van Andel of Stanford University and Jack Corliss of Oregon State took a few last breaths of the South Pacific air before closing the basketball hoop-sized hatch of the research submersible, Alvin. Their pilot, Jack Donnelly, then guided the 23-foot long craft down 9,000 feet towards the seafloor, away from the team’s research vessel Knorr and mother ship, Lulu. Ninety minutes later, the trio reached the bottom.
Six hours and forty-seven minutes later, they were back at the surface. When the science team extracted the first water samples taken from the seafloor, the entire lab on the Knorr was filled with a horrible stench: rotten eggs.
As terrible as this smell was, the discovery of hydrogen sulfide on the seafloor was a watershed moment in humankind’s understanding of the origins of life.
Six months later, on August 20, 1977, the Voyager 2 probe launched into space aboard a Titan IIIE/Centaur rocket from Cape Canaveral, Florida. The spacecraft sent back its first photos of Jupiter after twenty months in transit to the gas giant and after another two and a half months, it flew to within about 125,000 miles of Europa—one of Jupiter’s 64 moons. As raw images beamed back to Earth at a rate of one every 90 seconds, scientists, engineers, students and journalists sat glued to their seats staring at stunning images that revealed a white, icy world, criss-crossed with dark lines and a surface seemingly as smooth as anything thus far seen in our solar system.
Images and data received from Voyager 2 suggested that beneath that layer of water ice lies a liquid water ocean bigger than all of Earth’s oceans combined covering the entire moon—itself about the same size as our own moon.
Soon researchers began to wonder if, beneath that layer of ice at the bottom of thousands of feet of water and methane liquid, the seafloor there smelled like rotten eggs, too.
******
At first glance, space and sea might seem like opposite ends of a spectrum that defines extremely hazardous environments to life—or hazardous to our kind of life, at least. In space, there’s no air to breathe and thus, no air pressure to hold our skin and organs in place, maintain water in our bodies as a liquid versus a vapor, and keep oxygen circulating in our blood. A human can die within several seconds of exposure to the vacuum of space. In the oceans, an equally deadly environment exists, though at pressures up to several thousand times higher than what we’re used to—pressures that would crush us without the protection of a submarine or deep diving suit.
But both space and oceans share a lot in common. They’re both vast, almost entirely unexplored regions, compared to tiny us. And both have a lot to tell us about life.
The fact that life exists in the first place is rather incredible. Life could have very easily not existed. We haven’t yet found evidence for past or current life on any of the planets and moons in our solar system. We haven’t begun to scratch the surface on whether life exists outside our solar system, but we haven’t found it anywhere out there yet. And still, it’s everywhere here. We find life under highly acidic rocks, within massive glaciers, in the darkest chasms of the ocean. Try to find the most desolate, barren, inhospitable hell on Earth, and you’ll find life there, too.
Chris German is in the business of exploring some of Earth’s more hellish environments. A geochemist at Woods Hole Oceanographic Institution in Massachusetts, German leads research expeditions aimed at understanding the complex plate tectonics and chemical reactions taking place on Earth’s seafloors. By discovering the history of the planet’s shifting seafloor, he’s uncovering knowledge of the nature of extreme environments—the kind where life probably shouldn’t exist, but does.
At 8:00 a.m. Friday, January 6, 2012, German began perhaps the most promising research expedition of his 28-year career. He watched from the deck of the research vessel Atlantis as his team and crew totaling nearly fifty people embarked from Port Everglades, Florida. The last shipment of equipment had only narrowly arrived with half an hour to spare before the ship’s departure, culminating years of preparations for this expedition. Atlantis headed south, passed the Florida Keys in the late afternoon, and turned west, following a backwards S route that would take the team around the western end of Cuba to a stretch of ocean above a 70-mile long ridge in the seafloor called the Mid-Cayman Rise (MCR). Their target: new hydrothermal vent sites along the ridge that German and colleagues had explored and mapped during two prior survey expeditions in 2009 and 2011. Dubbed “OASES 2012: Return to the Cayman Rise,” this research expedition was chronicled by Julia DeMarines, a research assistant of the astrobiology curator at the Denver Museum of Nature and Science.
Hydrothermal vents happen where near-freezing water at the bottom of the ocean meets the scalding hot rocks of young ocean crust. At mid-ocean ridges like the Cayman Rise, magma is produced by a heat source that comes from energy released from the decay of radioactive materials deep inside the planet and also leftover heat from the Earth’s formation. When water and hot rocks meet, the now superheated water shoots up through cracks in the seafloor, creating plumes of hot gases and chemicals that can reach several hundred feet in height. The plumes are often black and sooty—the ocean version of a diesel train engine belching a steady stream of black smoke into the sky. This gives rise to the nickname black smoker. Miraculously, this mix of hot chemical-laden fluids and cold seawater creates conditions that form the ocean equivalent of a desert oasis.
These sites host communities of living matter that can be denser than any patch of life on land in the known biosphere and 10,000 to 100,000 times greater than the rest of the seafloor. Here, exotic tendril-like organisms called tubeworms can grow eight feet tall and clams can be a foot across. Normally, clams a third of an inch across are a century old. Yet, at some plumes, clams nine inches across are just ten years old. That means their growth rates are 300 times faster than those of clams anywhere else on the seafloor.
This is remarkable given that the pace of life on the bottom of most of the ocean is slooooow. Once, in 1968, the Alvin submersible sank to a depth of 5,000 feet after a cable attaching it to its mother ship snapped as the vessel was being lowered into the water. The three-person crew was able to scramble to safety, but they forgot to grab their bag lunches: three apples and three bologna sandwiches. These sat on the ocean floor for 10 months before a rescue crew was able to recover Alvin. Upon finding the lunches, the crew was amazed at how little they had changed—some even tasted the sandwiches. Salty, but fine, they said. It was as if the biological process of degradation had simply stood still.
Yet, life thrives at hydrothermal vents. They’re also diverse by location—just like distinct biogeographic regions on land. Life forms found in one ocean can vary significantly from those found in another ocean. The 2011 cruise had been especially productive in proving the amazing diversity of life near these extraordinary sites. German had personally witnessed the discovery of the first live tubeworm found near a hydrothermal vent in the Atlantic Ocean. Bigger still, that team had found chemosynthetic shrimp (which live off energy from chemical reactions from the vents instead of photosynthetic processes involving the sun) and tubeworms occupying the same site—perhaps the landlubber equivalent of finding a lion and a polar bear chewing on the same antelope in the middle of the Sahara.
Now the team was heading back to the MCR to collect the first physical samples from what some consider the most interesting collection of hydrothermal vents on our planet.
The MCR is home to the deepest known underwater volcano in the world and is unique among the world’s 42,000 miles of ocean ridges for its geologic diversity. “In a nutshell, it displays perhaps the broadest range of mid-ocean ridge geologic processes all active in the same place,” says German. The ridge is considered an “ultraslow” spreading ocean ridge, meaning it moves at a rate of less than an inch a year, as compared to the relatively speedy “slow” spreading ridges which move at just under two inches a year or “fast” spreading ridges which have been clocked at a blazing eight inches a year. The site is the home of three different types of vents, which are classified by their temperature and the chemical composition of the rocks that give life its fuel.
The first, Type 1, is common throughout the world’s mid-ocean ridge system and is characterized by high temperatures (sometimes more than 400 degrees Celsius) and “mafic” rocks from the Earth’s crust, meaning they are rich in magnesium and iron. Type 2 vents have similar high temperatures, but these vents are the result of reactions between seawater and far deeper rocks from the Earth’s mantle with even higher concentrations of magnesium and iron. These are classified as “ultramafic.” Type 3 vents, too, are ultramafic but have much lower temperatures. Since they’re hosted in rocks formed at even higher temperatures than what erupts at the seafloor today, they are directly comparable to material that erupted on the early Earth. As such, ultramafic vent sites are among the most exotic and captivating of hydrothermal environments to marine scientists.
Chief Scientist German had found evidence for at least three vent sites during his first trip to the MCR in 2009. Past experience in the southern Indian and Arctic Oceans had tempered expectations for discovery of new vents sites to no more than roughly one site for every 100 miles of mid-ocean ridge explored. Since the MCR was only 70 miles long, that first voyage was “probably the highest-risk expedition I have ever undertaken,” says German. The probability of finding even one vent site there was fairly low to begin with.
Thus, finding three sets of plume signals in those original water-column studies, indicative of three different types of venting on the same relatively small stretch of mid-ocean ridge was astounding, a bit like looking for a coffee shop and finding a Starbuck’s, a Dunkin Donuts and a Caribou Coffee on three corners of an intersection of a two-road town in the middle of a Nebraska cornfield. That’s why, in the world of hydrothermal vents, there’s more than one reason why this specific stretch of seafloor real estate is considered a “hot spot.”
Unfortunately, the team’s original discovery was followed closely by setback. The 2009 expedition was cut short by Tropical Storm Irene before the team could get up close to any of the prospective sites with its Remotely Operated Vehicle (ROV), Nereus. German had to wait until the 2011 opportunity to get a closer look, but this 10-day expedition was only intended to photograph and map the vent sites. No samples were collected. Both expeditions paved the way for the 2012 expedition, which would be the first to study each site in detail and to obtain actual samples of the rocks, water, tubeworms, and other critters in the vent communities.
German hopes that the samples from these wildly diverse vents, all within a small patch of mid-ocean ridge, will reveal secrets to the formation of the early Earth, and maybe while they’re at it, the origin of life itself. By studying how a living ecosystem can sustain itself in the deep, black ocean, far below the reach of the sun’s rays, German isn’t just classifying exotic worms and other animals in a quirky Twilight Zone setting—he’s looking through time.
“Just like the Hubble Telescope, pointed at the most distant points of the Universe, hydrothermal sites like these may become a sort of lens that allows us to view back into Earth’s distant past and explain how life originated on Earth,” says German.
And just like the Hubble Space Telescope, his expedition is funded significantly by the agency largely responsible for directing our eyes skyward: the National Aeronautics and Space Administration.
Duck Pin, We Have a Problem
Banking his Boston duck tour boat in a gradual U-turn on the Charles River, the gray-goateed helmsman and tour guide known as Duck Pin guided the vehicle through the glassy water toward the Longfellow Bridge, his bowling shirt flapping gently in the breeze. “And there, behind those buildings about three blocks down on Broadway,” said Duck Pin, “was the site where NASA wanted to build its Mission Control Center, right next to MIT in the Kendall Square area.”
(as published in MIT's Technology Review)
Banking his Boston duck tour boat in a gradual U-turn on the Charles River, the gray-goateed helmsman and tour guide known as Duck Pin guided the vehicle through the glassy water toward the Longfellow Bridge, his bowling shirt flapping gently in the breeze. “And there, behind those buildings about three blocks down on Broadway,” said Duck Pin, “was the site where NASA wanted to build its Mission Control Center, right next to MIT in the Kendall Square area.”
Several passengers nodded as they gazed up the Charles River embankment.
“If it wasn’t for Kennedy’s assassination, we would have been saying ‘Cambridge, we have a problem’ instead of ‘Houston,’” Duck Pin said. When the president died, “NASA decided to move Mission Control to Houston—conveniently in Texas, the home state of our new president, Lyndon B. Johnson.”
More heads nodded. Maybe they’d heard it before—the legend of the NASA Mission Control Center in Cambridge has been circulated in print and passes freely among professors and students in the hallways of MIT. It’s the story of a grand vision that never was—aborted, like so many other plans, hopes, and dreams, at the death of the young president from Massachusetts.
Problem is, the story’s wrong.
As it turns out, Kennedy’s death wasn’t really a factor in NASA’s Cambridge history. In 1961, Massachusetts politicians tried but failed to win a Cambridge site for the Manned Spacecraft Center (MSC), an engineering, training, and operations complex to complement launch operations facilities at Cape Canaveral, Florida. Despite pressure from Massachusetts governor John Volpe and Senator Benjamin Smith, NASA instead selected Houston. According to NASA’s James Webb, Kennedy had “intervened in no way to try to favor his own state of Massachusetts, or to rule it out of the game.” The MSC opened on March 1, 1962, nearly 21 months before Kennedy’s assassination.
On July 20, 1962, NASA announced the decision to locate its Mission Control Center at the MSC in Houston. That September, Kennedy gave his famous “We choose to go to the moon” speech before a crowd of 35,000 at Rice University in Houston. Construction on the new mission control facility began in late 1962, roughly a year before the president was killed on November 22, 1963.
The story of NASA’s history with Kendall Square may have come from confusion over a separate effort to develop a research and development center near MIT. As agency decisions were shaping Houston into the Space City, Massachusetts lawmakers were lobbying to bring to Cambridge a NASA field center meant to address the agency’s need for internal expertise in high-quality electronics.
In spite of local opposition (the project would force the removal of 94 local businesses), Kendall Square got the field center. NASA officially opened the Electronics Research Center in a new building at 55 Broadway on September 1, 1964. With the loss of the MSC (renamed the Johnson Space Center in 1973), the Electronics Research Center was Cambridge’s consolation prize.
But NASA’s stay in Cambridge proved short-lived when Congress and the Johnson and Nixon administrations cut NASA’s funding even before the first successful moon landing in 1969. Envisioned as the lead NASA center in electronics, the ERC had 950 employees at its peak in 1968 and was involved in hundreds of long-range research projects in such areas as microwave and laser communications, radiation resistance, and holographic information display. But on June 30, 1970, NASA moved out, and the next day the Department of Transportation moved in. NASA electronics projects were cancelled or absorbed by other NASA centers; many staffers transferred to the DOT.
So although it makes a good story to say that Tom Hanks (playing astronaut Jim Lovell) might have declared “Cambridge, we have a problem” during the movie Apollo 13, it seems Duck Pin was wrong about his NASA trivia. Today, the DOT’s Volpe Center at 55 Broadway stands as a reminder of Cambridge’s legacy with the space program, and of Kendall Square’s brief role in humanity’s first steps into the cosmos.
A Decade Inside the Olin Oval
Ten years after a prominent foundation dumped all of its money into the creation of a tiny undergraduate engineering school from scratch, how does it shape up?
There’s an oval in Needham, Massachusetts, where big ideas are taking shape. The oval itself isn’t that big, maybe 400 feet at its longest and 300 feet at its most narrow. Three buildings, curved to match the oval’s outer arc, surround a concrete walkway around the perimeter and an unspectacular patch of grass is bisected by walkways crossing through the center. A grassy mall stretches out radially from the space between two of these buildings, expanding in size, manicured to perfection. Two more unassuming buildings line the mall and the whole 70-acre campus is dotted with pines and fields, hemlocks and parking lots, showcasing the simple beauty of Eastern Massachusetts with a nod to the elegant traditional design of New England colleges.
That’s it. Five buildings and an oval: the layout of a campus designed to redefine engineering education in the United States for the 21st century.
Ten years after a prominent foundation dumped all of its money into the creation of a tiny undergraduate engineering school from scratch, how does it shape up?
There’s an oval in Needham, Massachusetts, where big ideas are taking shape. The oval itself isn’t that big, maybe 400 feet at its longest and 300 feet at its most narrow. Three buildings, curved to match the oval’s outer arc, surround a concrete walkway around the perimeter and an unspectacular patch of grass is bisected by walkways crossing through the center. A grassy mall stretches out radially from the space between two of these buildings, expanding in size, manicured to perfection. Two more unassuming buildings line the mall and the whole 70-acre campus is dotted with pines and fields, hemlocks and parking lots, showcasing the simple beauty of Eastern Massachusetts with a nod to the elegant traditional design of New England colleges.
That’s it. Five buildings and an oval: the layout of a campus designed to redefine engineering education in the United States for the 21st century.
The oval is the centerpiece of the campus for the Franklin W. Olin College of Engineering, a tiny undergraduate engineering college with about 300 students. Opened in the Fall of 2002, Olin College is led by Richard K. Miller, a tall man with a shiny head and a bushy mustache, advanced degrees from MIT and Cal Tech and a distinguished career across many fields of engineering. Miller is a passionate advocate for thinking big when it comes to the future of higher education.
"Olin has a dual mission,” Miller explains, sitting in his office on the second floor looking out on the campus from the tip of the oval. One is the “mission inside the oval” and that amounts to educating a small number of exemplary engineering innovators every year. The second “mission outside the oval” is to create a school that is different—not for the sake of being different—“ but for the purpose of becoming an important and constant contributor to the advancement of engineering education in America and throughout the world.”
It’s a big mission for a tiny school, inside and out. Named after industrialist, engineer, and philanthropist Franklin W. Olin, the college was created after the Olin Foundation decided to shift all of its $400 million endowment away from providing resources to existing engineering schools and into the idea for an entirely new one—officially chartering Olin College in 1997.
Founded with an emphasis on engineering practice over theory, Olin has a “do-learn” philosophy designed with a strong interdisciplinary tradition rarely seen in engineering programs today. Olin’s graduates learn the social, environmental, business, and political context of engineering along with traditional applied science courses—an approach that has already produced impressive results in its first ten years of classes.
Since opening its doors, Olin has graduated five classes and unleashed more than 250 engineering innovators out into the open seas of the workplace and academia. Its alumni include four Fulbright scholars, nine National Science Foundation Scholars, and leaders in international development, biotech start-ups and energy systems companies. Yet, beginning from a blank slate hasn’t come without setbacks and controversy. Olin’s project-based learning, no-tenure philosophy, technology entrepreneurship courses and free tuition principles have elicited both envy and skepticism from a highly-entrenched culture of engineering higher education.
And—as any start-up can attest—the growing pains have been formidable.
“There’s no more powerful force for conservation than having something to conserve,” Miller says, quoting Joseph B. Platt, founding President of Harvey Mudd College. “I’m hoping that somehow I can continue to inspire our faculty to see the value in holding the line on (the principle of continuous cultural change). Because in 20, 30, 50 years from now, this will make the difference on whether Olin falls into the same trap that every other university does or whether it continues to remain committed to improvement and change.”
While hopeful, Miller says it’s too soon to know how successful Olin will be at maintaining its innovative culture. “At the end of the day, you can’t write a memo from the president’s office that everything’s going to be different tomorrow. There has to be an appetite for this and a commitment from the community to try new things. The jury’s out.”
*******
“What is an engineer?” Also, “What does every engineer need to know? Don’t we need to know this before we can develop the curriculum here?” In his approximately 25 years of experience in engineering education before sitting down with a small cadre of visionaries charged with crafting Olin’s mission, to that point, Miller, 61, had never heard any of those questions seriously raised.
So when Olin’s founding faculty decided to start from scratch, they really wiped the slate clean.
Ten professors from varied backgrounds from physics to engineering, math, chemistry, and even music started by literally looking at the available definitions of engineering and discussing their own experiences in the field. Working from temporary modular housing units on the edges of the still-under-construction campus—the founding group settled on a simple definition of an engineer as “one who makes.”
The problem was, engineering education had drifted too far into the theoretical realm and away from actually creating engineers who could succeed at the practice of engineering. That was reality, they concluded. Their task, therefore, was to develop a curriculum that broke free from the traditional model of applied science teaching while still equipping engineering graduates with the right technical skill set to succeed as makers.
Sociable, with a gentle Midwestern voice that’s equal parts Carl Sagan and Ernie from Sesame Street, Miller resembles an older, taller version of Goose from Top Gun. Leaning back in his chair across from a polished, golden wood desk, he describes how a wealth of experience in improving undergraduate engineering education at the University of Southern California (USC) and the University of Iowa helped shape his vision for Olin.
Miller had an epiphany in teaching while he was a professor in aerospace engineering at USC in the 80’s. He solicited requests for technical problems local aerospace companies were working on that could be used as hands-on, semester-long projects for the students.
One of the early trials involved an early design of what would become the International Space Station. The problem facing engineers at Martin Marietta was in the design of a structural coupling between pressurized tubes within the donut-shaped station. Essentially, they needed a coupling that could expand without twisting.
The student team came up with an idea that used a system of pulleys and cables to solve the twisting problem. The solution impressed Martin Marietta engineers, who had basically come up with the same solution—only theirs had resulted in a $200 million patent and had won them the contract over rival aerospace giant Boeing.
Miller continues: “So the kids came up to me after this presentation and they said, ‘Dr. Miller, we have a couple questions for you. … How do you make money from ideas like this? There’s nothing in my engineering courses that says like—‘$200 million. What would you do?’ Oh and by the way I’m going to graduate in about a month and I have this employment offer and right below this signature there’s this paragraph about intellectual property and stuff like that. So if I dream about things in the shower while I’m working for them, they own this? Is that Ok for me to sign?’ And I’m saying: uh-oh… we’ve got some work to do.”
*******
That experience started then-Professor Miller on the track to re-thinking engineering education. Yet, not everyone at the time was as willing to change as he was and Miller found he’d have to leave USC to implement some of his ideas.
In 1992, Miller was named Dean of Engineering at the University of Iowa and he set about righting some of the wrongs he’d seen while at USC. He started by creating an opportunity for engineering students to take business management and entrepreneurship classes alongside business students at the university. After hitting resistance initially about engineering students not having enough experience in economics to contribute, Miller and the rest of the faculty were immediately impressed with the creativity and commitment of the engineers.
“It didn’t matter that they didn’t have ECON 101—they were just too bright, and too committed and they could answer the technical problems that needed to be done in the consultant part of the work,” says Miller.
The students came back to the college of engineering and asked to take more business classes. But at the time, they were only allowed to take one elective and they’d just taken it. The curriculum wasn’t flexible enough to allow any other non-engineering courses.
The paradox between the passions he saw in his students and the rigidness of the curriculum at Iowa and USC inspired Miller to make Olin’s curriculum open, hands-on and interdisciplinary. Olin students can cross-register for business and liberal arts classes at neighboring Babson College, Wellesley College, or Brandeis University (one popular option is “Harry Potter and Politics” at Babson—no joke). They’re involved in challenging hands-on projects starting on day one of freshman year, allowed to study abroad during their junior year and still graduate in four years, must start and run a business before graduating, are required to “stand and deliver” at the end of each semester to present some aspect of their academic work in public, and must complete a year-long senior design project of a real-world problem sponsored by industry.
And so far, students are happy. In the 2010 edition of Princeton Review, Olin College is ranked among the top 20 universities in the U.S. in 14 different categories, including Best Classroom Experience, Best Quality of Life, and Happiest Students.
To Miller, rankings don’t mean everything. But they help validate his approach in upending the traditional philosophy towards engineering education. He realized “that engineers in fact don’t start with the differential equations—they end with the differential equations. It’s the ideas that are hard to come up with. And that the ideas have to be consistent with physical law—that’s where you add value—but this is much more of an artistic event than it is a scientific event.”
*******
Perhaps one of the biggest hallmarks at Olin is the stubborn attachment folks here need to have to the concept of continuous change.
“I think the idea and the way we express it—no one here at Olin has tenure,” says Miller. “And nothing at Olin has tenure. So don’t fall in love with it. Because we should be willing to let go of the steering wheel if there’s a better idea.”
It’s the nothing has tenure philosophy that really gets Miller revved up. “Let me just tell you some of the stereotypes which I hear all the time, that I don’t think any of us in higher education has taken seriously enough to develop a persuasive, coherent, well-researched response to. One of them is: tenure is a huge evil, it is the cause for a lot of the country’s underperformance in education, that it encourages deadwood, that it’s just completely indefensible.”
To ensure the culture at Olin doesn’t get too comfortable and stuck in its ways, Olin has a policy of periodic curricular review: every seven years the curriculum is re-designed.
But the policy of institutionalizing the blank slate has been easier said than done. As Olin has fought through its growing pains (during its inaugural semester, a group of dazed, over-worked students marched into the president’s office complaining of the workload, resulting in a moratorium on classes), the school has struggled to establish and maintain its unique identity. In some ways, maturity has brought with it aspects of academic conservatism Olin was founded to resist.
“In 1999 when we first arrived, we had nothing to conserve,” says Miller. “So we were really—every seven years is a great idea! Maybe we should make it every 3 years! Now that we’re 10 years later, we have stuff that some of our faculty members, and the rest of our community too, would not imagine ever throwing away. We think these are the reasons why we’ve been successful. So why would you ever want to throw that away? So guess what? If you hold a mirror up, we’ve become conservative. And I think there’s way more resistance to this idea in 2011 than there was in 2004. So it’s changing.”
Erika Swartz is a senior in Materials Engineering at Olin. Full of energy and heavily involved in Olin’s growth as a NINJA mentor (NINJA = Need Information Now? Jusk Ask) and as a project manager of her senior design project, Swartz admits Olin’s growth has not come without setbacks.
“Olin is kind of in puberty,” says Swartz. “We’re no longer brand new. We’re starting to develop these institutional traditions and we still have to define what our place is going to be and what our role is going to be. And I don’t know that we’ve done that yet.”
Constant feedback is Olin’s strategy for checking its gauges as it matures and tries to avoid conservatism. That involves a heavy dose of polling.
“Our students wind up being polled probably more than any other student body in North America,” says Miller. “And after awhile there’s sort of polling fatigue.”
Feedback, to Miller, is a vital component for an organization to resist complacency. “At its heart,” Miller says, “continuous improvement requires an authentic personal commitment to getting better. I think of it this way. I don’t know of a single Olympic athlete who doesn’t own a stopwatch. Ok? How are you going to know if you’re competitive in running the mile if you don’t even know what your time is in the mile? How are you as a university going to know if you’re getting better unless you’re measuring something?”
*******
So what is Olin’s stopwatch?
Olin maintains an internal institutional feedback system, says Miller. It’s called the Olin Metric Project. He stands up and grabs a thick binder from one of the many bookshelves lining his office and opens it up on the table. He points to a series of gauges indicating categories of metrics on one page in the middle of the binder that look like fuel gauges on a car’s dashboard console. On first glance, the gas tanks look like they’ve been topped off in nearly every category—attracting students, providing the right experiences, and ultimately, “educating engineering innovators.”
But there are signs financial problems loom. One category—financial resources—comes in at more like two-thirds full, clearly the lowest rating documented by the Olin Metric Project. Miller explains that the economic crisis is to blame. “We dropped over a hundred million dollars in the endowment, we’re more dependent on the endowment than other institutions. Financially, that’s caused some changes in what we’re trying to do,” he says.
Financial matters have been an area of constant concern during Olin’s first ten years. Originally, Olin’s founders wanted to provide free tuition to every student, which they were able to do up until 2009 when they were forced to scale back to half-tuition scholarships for every admitted student (worth about $80,000 per student). Founders also wanted to install a “study away” requirement that would require every student to spend a semester studying outside the U.S. at no extra tuition cost. This was also scaled back to the point where now Olin students are given the flexibility in their schedules to study away, but need to cover the additional cost of the international program on their own. So far, only 20-25% of each of Olin’s five graduating classes has chosen to participate.
Beyond financial concerns, Olin has fought the notion of being “too innovative” in its approach and not providing students with the background to succeed in traditional engineering roles. Constance Bowe is a senior consultant for Partners Harvard Medical International, which advises medical schools on education reform all over the world, and author of a 2007 case study on Olin College. She uses Olin as an example to medical faculty for re-thinking med school education—but not without caution.
She points out that in adding new interdisciplinary courses in business and design, the danger is in losing foundational knowledge and creating engineers who aren’t prepared for competing in a world based in traditional assessments. “If you spend a lot of focus on these emerging competencies that people are recognizing are essential to do the kind of professional work they have to do,” she says, “you are taking some time away from those building blocks, those scientific facts.”
Bowe found Olin’s assessment approach to be one of its most valuable assets to ensuring that foundational knowledge isn’t lost. Instead of relying solely on quantitative data (as most people with technical backgrounds—as well as most technical institutions—are most comfortable doing), she says Olin’s founders “had a very intriguing way of designing an assessment plan for their school.”
She says “they had actually created a culture at Olin where assessment was actually valued by everybody who had to participate in it.” That culture, combined with regular descriptive feedback, has helped Olin’s faculty fine-tune their curriculum.
“The subjective information is actually telling you if something’s good—what it is. Or if something’s not so good—how it might be better, what the problem is. Where the numbers don’t tell you any of that,” says Bowe.
Miller feels that despite some road bumps and the difficulty in quantifying the success of Olin’s grand vision, the small college is still on the right track.
“How do you take all of those metrics and decide that they amount to what we call a ‘green’? I don’t have an algorithm for that,” Miller explains. “There’s judgment here. And in spite of the fact that engineers are always skeptical about judgment, I’m skeptical about not having judgment at the end of the day. So I’m sure it’s the right thing, but our opinions will change as we get more experienced.”
*******
Swartz, for one, has seen the success of the Olin Experiment firsthand and says she wouldn’t trade her four years at Olin for anywhere else. She says the value of the school—and what really sets Olin students apart from others—is the emphasis at Olin on solving challenges with undefined parameters and applying creativity and hands-on skills to come up with solutions to improve lives.
In other words, solving real problems in the real world.
Or, in Olin’s founding faculty’s definition, becoming one who makes.
“One really big thing (that makes Olin students different) is being comfortable with uncertainty and open-ended problems,” says Swartz.
She says that where students from traditional engineering schools might get frustrated with gargantuan, open-ended problems (determining what’s halting the educational pipeline in Uganda from educating citizens past a sixth grade reading level, for example), Olin students thrive.
They’re taught from their first days on campus to “find out a little bit more about the problem space and decide ‘ok, what is the actual question that I’m trying to answer? Within this really broad problem statement, what is the specific small problem I’m trying to solve?’” says Swartz. “And I think that’s a really important real-world skill. To take something complex and break it down into a solvable unit, using the knowledge you have.”
In terms of redefining undergraduate engineering education for the 21st century, leaders in higher education certainly face a complex problem that will need to be broken down into one solvable unit at a time.
At Olin College, among the tree-lined hills and within the visionary minds of Richard Miller and his brave un-tenured faculty in Needham, the shape of that unit is an oval.
On the Playground with the Bullies of Science
A review of The Essential Engineer: Why Science Alone Can’t Solve Our Global Problems, by Henry Petroski (274 pages. Knopf, 2010. $26.95)
If would-be pocket-protecting scientists were the kids that received wedgies on the playground and were nearly forced into malnutrition by bullies stealing their lunch money, I wonder what would-be engineers endured in Henry Petroski’s school.
“Engineering can be as much of an assault on the frontiers of knowledge as is science,” asserts Petroski in The Essential Engineer, sounding the battle trumpet of engineering. A professor of civil engineering at Duke University, Petroski’s out to get engineers some respect. He’s tired of bully scientists hogging the spotlight of public esteem and relevance.

A review of
The Essential Engineer: Why Science Alone Can’t Solve Our Global Problems
, by Henry Petroski
274 pages. Knopf, 2010. $26.95
If would-be pocket-protecting scientists were the kids that received wedgies on the playground and were nearly forced into malnutrition by bullies stealing their lunch money, I wonder what would-be engineers endured in Henry Petroski’s school.
“Engineering can be as much of an assault on the frontiers of knowledge as is science,” asserts Petroski in The Essential Engineer, sounding the battle trumpet of engineering. A professor of civil engineering at Duke University, Petroski’s out to get engineers some respect. He’s tired of bully scientists hogging the spotlight of public esteem and relevance.
A writer of fourteen previous books aimed at communicating the engineering profession to the general public, Petroski is often referred to as “the poet laureate of technology.” And if you’re looking to fill an open spot at your weekly poker meeting, you might consider inviting him. A prospective reader need look no further than the front cover to see his cards in the subtitle: Why Science Alone Will Not Solve Our Global Problems. This reveals two important aspects about your potential poker foe in Petroski that come up repeatedly in The Essential Engineer and are pills you’ll just have to swallow to get to his message: a) he’s got a beef with science and b) he’s got a flare for the obvious.
First off, is there anyone who’d argue that science alone will solve our global problems? I’ve met scientists paled to ghostly hues by entire lifetimes spent beneath the faint hum of vent hoods and LED track lighting within the belly of a basement laboratory, emerging only occasionally to scratch their heads and squint quizzically at the bright yellow ball in the noonday sky and even they’d be hard-pressed to argue for Science as Savior of all things worldly and mortal.
With scientific literacy in its current state, I imagine many an American citizen to read this subtitle and think, “wait, nobody told me science was supposed to solve all our problems! Here all this time I’ve been buying stock in Facebook!”
My own misgivings about the subtitle and the state of American scientific literacy aside, I found the engineer in me cheering right along with Petroski on his quest to elevate the unsung engineer in a world seemingly aligned to praise the great scientist. “Engineers tend to crow so little that they seldom get recognized for their work,” writes Petroski. In The Essential Engineer, he volunteers to do the crowing.
Petroski writes of engineering as an organic process that doesn’t need to wait for science to give a complete understanding of phenomena before setting off to change the world. He gives vivid examples—Thomas Edison enlightening the American industrial machine, Guglielmo Marconi sending wireless telegraphy over the ocean for the first time despite not possessing an understanding of electromagnetism, the Wright Brothers designing their aircraft before a theoretical basis for aerodynamics was established—that he uses to strengthen the case that engineering should be its own pillar of equal height to science in the foundation of modern civilization. This is where Petroski’s extensive technical experience and curious mind for the stories behind technology shines through.
He goes on to claim Einstein as an engineer at heart, linking his humble patent office beginnings with a fascinating account of one of Einstein’s own failed patents (written with fellow physicist-inventor Leo Szilard) on a refrigeration system using butane as a refrigerant over natural gas, which had been leaking from compressors with occasionally fatal consequences. By Einstein’s example, Petroski develops the idea that patents have served as a vital cog in the iterative process of engineering. Again drawing comparisons to science, Petroski writes, “Each new patent is akin to a new hypothesis.”
Petroski continues to explore the shared intellectual heritage and symbiotic relationship between science and engineering through three chapters of explanation into the history of the linear model of research and development in the United States, which traces its roots to American engineer and first presidential science advisor during World War II, Vannevar Bush. “Just as science is a never-ending quest to uncover the mysteries of the universe,” writes Petroski, “so engineering is the never-ending pursuit of a better system, including how the nuts and bolts will interface with the dollars and cents and the supply and demand.”
Yet at times, Petroski indicates in not so subtle terms that the pillar of engineering should stand even taller than that of science. The big, hairy, nasty problems in the world like climate change, alternative energy, and natural disasters need engineers and engineering to play a leadership role, he argues. That’s because engineers, like scientists, are in the business of dealing with risk and uncertainty. But unlike scientists, engineers are equipped to cut across disciplines to apply creative solutions to these problems using their understanding of risk and uncertainty, whereas scientists give us only warnings.
This is where Petroski’s optimism at having a winning hand might get the best of him. In over-simplifying the shortcomings of science, Petroski seems to overstate the promise of technology and design. Quotes like: “The engineer is a worldly scientist,” and “if we wish to solve real and pressing problems, we should focus on the development end of R&D” merely amplify the biased tone throughout the book, further alienating the cause he says he wants to champion—that scientists and engineers actually need to work together to solve complex challenges.
Maybe it was The Essential Engineer’s genesis from a scattering of Petroski’s regular columns in American Scientist, the magazine of Sigma Xi, the Scientific Research Society, and ASEE Prism that causes it to lose focus. Tangents into the design of speedbumps, the Blackberry’s effect on the blurred line between work and leisure, and the design of the ampersand are distracting allegories that dilute his point: here, he wants to make a claim for increased emphasis on engineering’s role in solving society’s problems and ends up with an interesting but disconnected list of mundane things engineers happen to do.
Other segues into the realm of the distractingly obvious include the assertion that “there are no easy solutions to tough problems” and “[engineering] is a journey with frequent stops, much backtracking, and many redirections, but never a truly final destination.” Couldn’t one say the same about, oh, just about anything? Skateboarding? Learning a new language? Attempting to unlock the ultimate answer to the origins and fate of the universe?
These are minor qualms that could be overlooked if they didn’t recur repeatedly throughout the book. Instead, they discredit the author’s renowned credibility as an international thought leader in the field of engineering. Nowhere was this more clearly evident than in the penultimate chapter, when Petroski punctuates his attack on science in a strange and counterproductive manner. He writes about the Grand Challenges issued by the National Academy of Engineering, which are listed as follows:
• make solar energy affordable
•
• provide energy from fusion
•
• develop carbon sequestration methods
•
• manage the nitrogen cycle
•
• provide access to clean water
•
• restore and improve urban infrastructure
•
• advance health informatics
•
• engineer better informatics
•
• reverse-engineer the brain
•
• prevent nuclear terror
•
• secure cyberspace
•
• enhance virtual reality
•
• advance personalized learning
•
• engineer the tools for scientific discovery
•
This point could be a great opportunity to expound on the necessity of shared commitment with scientists to resolve these and other difficult challenges in the world. Instead, Petroski chooses the last several words of the chapter to call attention to the fact that “the up-front verbs used in the bulleted list of challenges are not the scientific verbs of discovery and understanding; they are active calls for creative achievement. They say ‘engineer’ and they challenge engineering.”
Never mind that these challenges were developed by the National Academy of Engineering.
Ultimately, the relevance of the topic and the richness of the stories of The Essential Engineer make it an important book in a relatively small niche market of popularizing engineering literature. Petroski’s message rings a tune that a technologically-dependent society needs to hear. And despite a grudging tone suggesting deep wounds on an ego inflicted by a society that would rather side with the playground bullies of science fame and prestige than mettle with the tinkerers of worthy widgets and practical panache, Petroski’s message comes across loud and clear:
Engineers are essential, yes.
Entropy and the Kitchen Sink
Is your sink full of dirty dishes right now? Mine is. Well, it isn’t full, but it’s got a few items in it I’ve been putting off washing since yesterday. There’s a plate I had pizza on last night, the knife I used to cut the pizza, a fork I used to pick sausages from the pizza when it was too hot to pick up the whole thing, one coffee cup shaped like a cow, and the little grease-catching tray thing from my George Foreman grill—a hold-out I missed during the last Great Kitchen Cleanup of 2012 sometime last week.
Is your sink full of dirty dishes right now? Mine is. Well, it isn’t full, but it’s got a few items in it I’ve been putting off washing since yesterday. There’s a plate I had pizza on last night, the knife I used to cut the pizza, a fork I used to pick sausages from the pizza when it was too hot to pick up the whole thing, one coffee cup shaped like a cow, and the little grease-catching tray thing from my George Foreman grill—a hold-out I missed during the last Great Kitchen Cleanup of 2012 sometime last week.
I’ll do dishes a couple times a week (on a good week), but almost never right after a meal. I let them build up a little before I give in. I think that’s normal. So normal that physicists even have a term for this practice of hygienic procrastination. They call it entropy. It’s the tendency for disorder to steadily increase in a system, or to think of it a different way, for the amount of useful work to decrease in a system over time. Some say it may eventually bring about an extreme state of critical sluggishness in the universe, when disorder has reached such epic levels that all the bits and pieces we’re made of, all the stars and planets and black holes and galaxies have completely degraded to a state of universal, equally distributed heat and matter. When life as we know it ceases to exist.
The ultimate sink full of dirty dishes.
(Don’t worry, we technically don’t need to worry about this breakdown for another 10^100 years. That’s a one with a hundred zeroes behind it, otherwise known as a googol.)
It isn’t such a bad thing, though. The fact that disorder increases in a system is a critical reason we’re alive in this universe to begin with. It really refers to the physical law that energy is always trying to create an equilibrium. It’s the same thing with the pizza I ate last night. The pizza is hot when it comes out of the oven, but eventually, it’ll get cooler as the heat—also a form of energy—dissipates into my kitchen air (or the roof of my mouth), in an effort to reach the same temperature as the rest of the surrounding system.
And this is irreversible—a crucial aspect of the nature of time in our universe. The pizza will not spontaneously become hot again. You can’t go back in time to un-bite into that scalding slice. Once the system has been balanced, that’s it. The potential for energy to be exchanged—or do work—in our enclosed pizza/kitchen air system has gone down.
You can re-heat the pizza, but that requires energy from somewhere else—electricity converted into heat or gas burned to create a flame maybe—to make it hotter. But eventually, that pizza will be just as cool as the air around it once again.
This irreversible balancing of energy is being played out across stages all over the universe, and since the total amount of energy in the universe is constant, this means that nature is trying to get us into a state where no energy exchange can happen. Perfect equilibrium. A state where the potential for work has disappeared completely.
Today, we use the term “entropy” loosely to describe all kinds of phenomena. Corporate entropy is the energy an organization wastes in dealing with bureaucratic red tape and inefficiency. Economic entropy is the permanent degradation of natural resources (and thus, energy). Even psychologists have an entropy. Their’s is related to the stubbornness of one’s personality and expresses the decreased capacity for dealing with sudden change.
But in physics, entropy is this continuous trend toward an overall decrease in the ability for work to get done in the universe.
So is Mother Nature encouraging us to just let those dishes pile up in our sinks?
On the scale of a googol, probably yes. In terms of turning my kitchen into an evolving ecosystem of exotic bacterial molds, fungi, and lines of marching ants, I’ll keep doing the work to wash my pizza plates and grease trays.
But if they pile up from time to time, that’s okay. I’ll just blame physics.